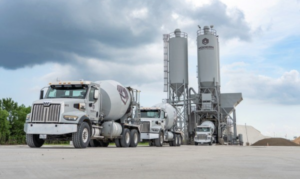
Resources
Read educational blogs on concrete trends and insights, case studies about customers’ experiences, or informational videos. Get access to webinars and learn concrete terminology, all at one place.
Read educational blogs on concrete trends and insights, case studies about customers’ experiences, or informational videos. Get access to webinars and learn concrete terminology, all at one place.
The construction industry is going through some big changes. Robots, AI, and smart sensors are starting to show up on jobsites, helping crews work faster, safer, and with more accuracy than ever before. Whether it’s…
Hot weather concrete placement presents a unique set of challenges, particularly for mass concrete elements. Without proper planning and temperature control, warm conditions can affect the concrete and shorten the service life of the structure….
Construction dispute resolution is a crucial aspect of construction project management, aimed at identifying, addressing, and resolving conflicts that arise throughout the project lifecycle. An effective dispute management process ensures that projects are completed on…
In this episode of The Construction Revolution Podcast, we sit down with Melvin Newman of PataBid. Melvin co-founded PataBid in 2018 after 15 years working in the construction industry as an estimator and project manager, where he was constantly looking for ways to optimize his roles using automation and technology.
In this episode of The Construction Revolution Podcast, you’ll hear from industry experts and leaders on the challenges of sustainable construction, and how the industry can work together to overcome these roadblocks. This panel discussion was originally recorded in March 2022, at the NetZero Construction Conference.
In this episode of the Construction Revolution Podcast, we sit down with Dobrila Rancic Moogk, VP of Marketing, at Giatec Scientific. Dobrila has over 25 years of experience in the tech industry in marketing, product management, and research.
On-Demand WebinarEnhancing Concrete Durability Using Smart Technology https://youtu.be/ay7IvJrC-7gIf you have questions during this on-demand webinar, send an email to support@giatec.caDownloads SmartRock Brochure SmartRock Quick Start Guide DescriptionThis webinar explores the challenges of concrete durability in…
Join our webinar to learn about construction efficiency, temperature match curing, SmartRock technology, and ready-mix concrete consistency.
Join Giatec’s Manager of Engineering Solutions, Icaro Mariani, to learn more about post tensioning and how temperature and maturity sensors can help on your next post tensioning project.
The concrete game has changed. Find out what self-calibrating sensors can do for you with Giatec’s SmartRock® Pro.